Topics and Learning Outcomes
Topics | After reading this Unit and completing the assigned readings in Physical Geology and the associated exercises and questions, you should be able to: |
---|---|
2-1 Weathering and soil |
|
2-2 Sedimentary rocks |
|
2-3 Metamorphic rocks |
|
2-4 Geological time |
|
Reading
Read Chapters 5 to 8 in Physical Geology.
2-1 Weathering and Soil
Before we talk about weathering, it’s important to first think about how it fits into the rock cycle (Figure 3.2 in your textbook). Since rocks cannot be weathered at all if they are still buried beneath other rocks, the key precursor to weathering of any kind is uplift, the removal of overlying material, and exposure. Plate tectonic processes, especially plate convergence and continental collisions, are primarily responsible for uplift. Once uplifted to form mountains, rock can be eroded and weathered tens to hundreds times faster than if it remained part of a flat plain (see Figure 2-1 and Figure 5.6 in your textbook).
Enlarge
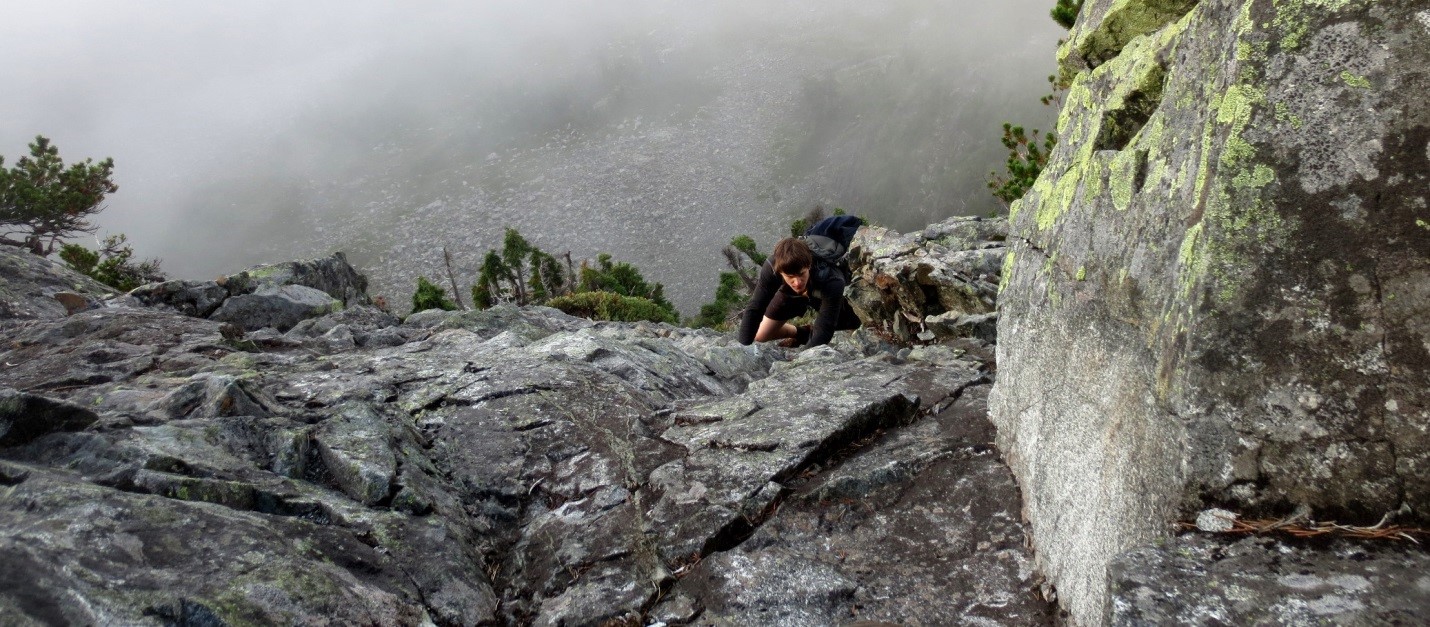
© Steven Earle. Used with permission.
Section 5.1 describes various types of mechanical weathering. Make sure that you understand the mechanics of these processes, and the types of environments in which each one might predominate. For example, freeze-thaw-related weathering requires repeated freezing and thawing, so, contrary to what you might first think, it doesn’t play a significant role during the fall, winter, and spring in the far north. It doesn’t play any role at all in regions where freezing is rare.
Completing Exercise 5.1 will give you some practice in identifying the processes of mechanical weathering.
Chemical weathering (Section 5.2) occurs because minerals are only stable within a restricted range of temperatures and pressures. Most minerals form at high temperatures and high pressures, and when they are exposed at the surface, they become unstable and begin to change. The main catalysts for this change are the weak carbonic acid present in all surface water (see the first equation in Section 5.2) and the abundance of oxygen of the atmosphere.
Since feldspar is the most common mineral of the crust, the most important chemical weathering process is the transformation of feldspar to clay. Clay minerals are much softer and weaker than feldspar, so a rock that is altered to clay (such as the granite in Figure 5.9) is much more susceptible to mechanical weathering that it was before. This phenomenon applies to all types of chemical weathering, and it works both ways as well. The disintegration of rock by mechanical weathering creates much larger surface areas for the processes of chemical weathering.
Iron is a common mineral in the Earth’s crust, and like many other elements, iron can exist in different “oxidation states.” The iron in a nail is present in the “reduced” form (ferrous iron), but when exposed to water and oxygen, it converts to the oxidized form (ferric iron) as illustrated in Figure 2-2, and as described in the textbook. The oxidation process of iron from minerals like pyroxene (FeSiO3) and pyrite (FeS2) releases acidity (H+ ions) into the environment, which can be responsible for a serious type of pollution known as acid rock drainage.
Enlarge
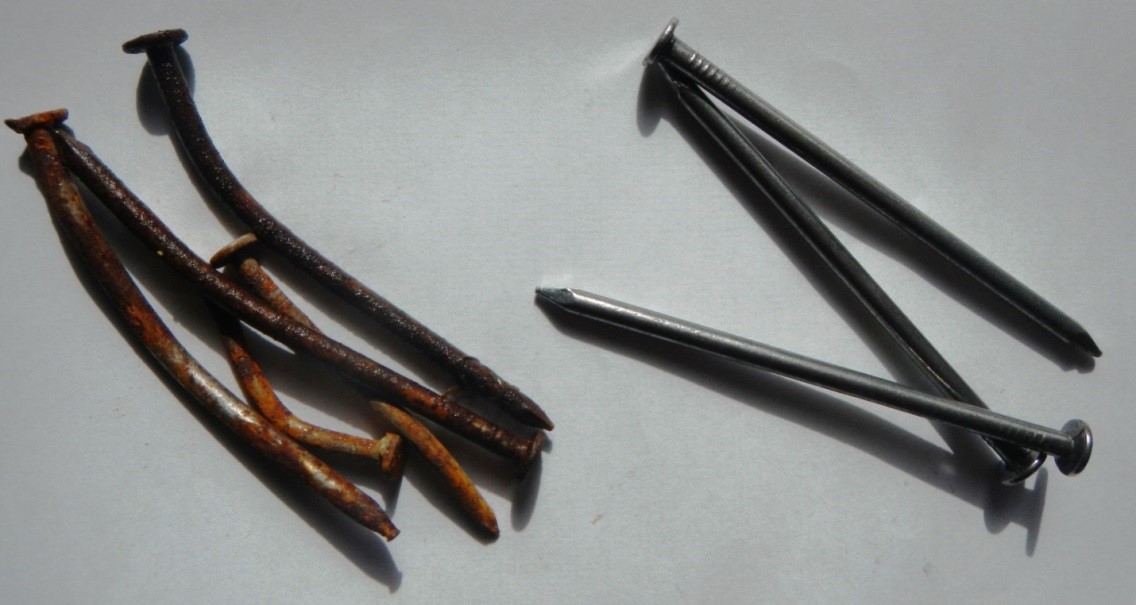
© Steven Earle. Used with permission.
Some minerals dissolve completely during weathering, and the most common example is calcite (CaCO3), the main constituent of the rock limestone. Limestone caves and other karst features form when parts of limestone bodies are dissolved away.
Completing Exercise 5.3 will give you an opportunity to review the different types of chemical weathering.
Weathering rates differ in different climates. In general, weathering is enhanced at higher temperatures and with increased moisture levels, but as noted previously, freeze-thaw processes require frequent transitions from freezing to thawing, and from thawing to freezing to be effective.
Exercise: Think about what type of weathering would be most prevalent in the following locations: coastal Brazil, the Gobi Desert in Mongolia, and the BC-Alberta Rockies.
Most of the common silicate minerals are significantly susceptible to chemical weathering, but one significant exception exists. At surface temperatures, the mineral quartz is essentially unreactive, and so while it breaks down mechanically—typically into sand sized grains—these grains can last for millions of years in the surface environment. This explains why the sand of most rivers, beaches, and deserts is dominated by the mineral quartz, and also why quartz is the dominant mineral of most sandstone. Table 5.1 in the textbook is an overview of the weathering products of different minerals. Many of the minerals, other than quartz, weather to clay minerals and to ions in solution, so it’s not surprising, as stated in Section 5.3, that quartz grains, clay minerals, and ions in solution are the most common products of weathering.
Completing Exercise 5.2 will give you some practice in interpreting weathering and transportation processes based on observations of the characteristics of sand grains.
Section 5.4 includes a discussion of the formation of soil, which would not exist without weathering. As shown on Figure 5.14, the main mineral components of soil are sand (mostly quartz), silt (a mixture of fine-grained quartz and other minerals), and clay minerals. All of these components are the products of mechanical and chemical weathering, and many of them are rich in chemical nutrients—such as phosphorous, potassium, magnesium, and calcium—that plants need to thrive.
The textbook includes a summary of the important controls over soil development. Please make sure that you understand why climate, parent material, slope, and time are important. Also, have a look at the discussion about soil horizons, which typically develop because of the downward percolation of water and the elements that it carries in solution. Under many circumstances, well-defined soil horizons will develop, including the O, A, E, B, and C horizons shown in Figure 5.16. See if you can identify some of those horizons in the profile in Figure 2-3.
Enlarge
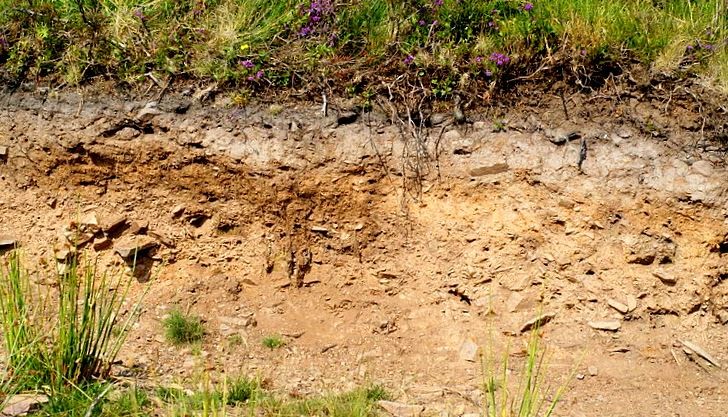
Egger, J. (2013). Heather soil profile from Badgworthy Hill, Devon, England (Image). Wikimedia Commons. Retrieved from: https://commons.wikimedia.org/wiki/File:Heather_soil_profile,_Badgworthy_Hill,_Devon,_England_(close-up).JPG
In dry areas, where evaporation is a dominant factor, water may move upward through the soil profile most of the time, and typically bring calcium ions with it. This process can lead to the development of a calcite-cemented soil known as caliche.
As noted at the start of Section 5.4: “… we need to take care of our soil.” However, many of our agricultural and other practices have been very hard on what should be considered our most precious resource (see Figures 5.17 and 5.18 in the text). It’s a good idea for all of us to be aware of how to take care of soil, even if we don’t dig it ourselves.
Section 5.5 examines the regional distribution of soil types in Canada. It’s not critical for you to know that distribution, but it would be worthwhile for you to complete Exercise 5.4 to gain some understanding of the factors that are important to soil development.
Section 5.6 includes a discussion of the relationships between weathering and climate change. Quickly read through this section, and we’ll cover these concepts in more detail in Unit 5.
Complete the questions at the end of Chapter 5. The answers are provided in your textbook.
2-2 Sedimentary Rocks
As described in the introduction to Chapter 6, sedimentary rocks make up the right-hand side the rock cycle, which involves the transportation, deposition, and lithification of the products of weathering and erosion. These products include sediments of various types that range from boulders to clay, and ions in solution. The resulting sedimentary rocks are known as clastic if they are made up mostly of rock and mineral fragments (i.e., clasts) or chemical if they are made up mostly of material that was carried to the depositional site in solution (as ions).
Sediments are classified by size because their transportation by water or wind is primarily dependent on their size. Their classification, described in Table 6.1, is based on a doubling scale in which each category is twice as big as the one previous. The sand grain classes, for example, go from 1/16th to 1/8th, ¼ , ½, 1, and 2 mm. You don’t need to know or memorize the Wentworth Scale, but it is worth remembering this: everything smaller than 1/16th mm is finer than sand (and particles that small don’t feel gritty between your fingers), and everything bigger than 2 mm is coarser than sand.
Completing Exercise 6.1 should help you to understand classification of grains. If you can visit a beach of some type, determine if the grains are sand-sized, or finer or coarser than sand. The grains may be different sizes in different parts of the beach. Take some extra time to determine if the grains are well rounded or not (which is a lot easier if they are bigger than sand). If you can’t get to a beach right now, think about some beaches or river bars you have visited, and try to remember whether the clasts were sand-sized, pebble-sized (up to about 6 cm), or bigger.
Figure 6.3 shows the forces on a grain of sand in water. As gravity forces it down, friction is the resisting force that pushes it up. If you drop a pebble and a grain of sand in air, the pebble will hit the floor first, but only by a fraction of a second. Drop the same pebble and sand grain into a metre of water, and the pebble will hit the bottom several seconds before the sand grain. In both cases, the gravitational forces are the same, but the frictional forces are quite different because water is so much more viscous than air. This principle is important because it is the rate at which particles settle through water (or air) that determines the extent to which they can be transported in flowing water (or wind).
Of course the other key factor in this regard is the velocity of the water (or air). The faster the water flows, the more turbulent it will be, which contributes to eroding sediments and keeping them in suspension. Also, the faster the water moves, the farther the suspended sediments will travel before they settle to the bottom. Look carefully at Figure 6.5 in the textbook to understand the transportation of (a) the larger sediments in a stream by traction and saltation, (b) the smaller sediments in suspension, and (c) ions in solution. Almost always, except at very low velocities, some particles are being eroded and some deposited at virtually every location along a stream (Figure 2-4).
Enlarge
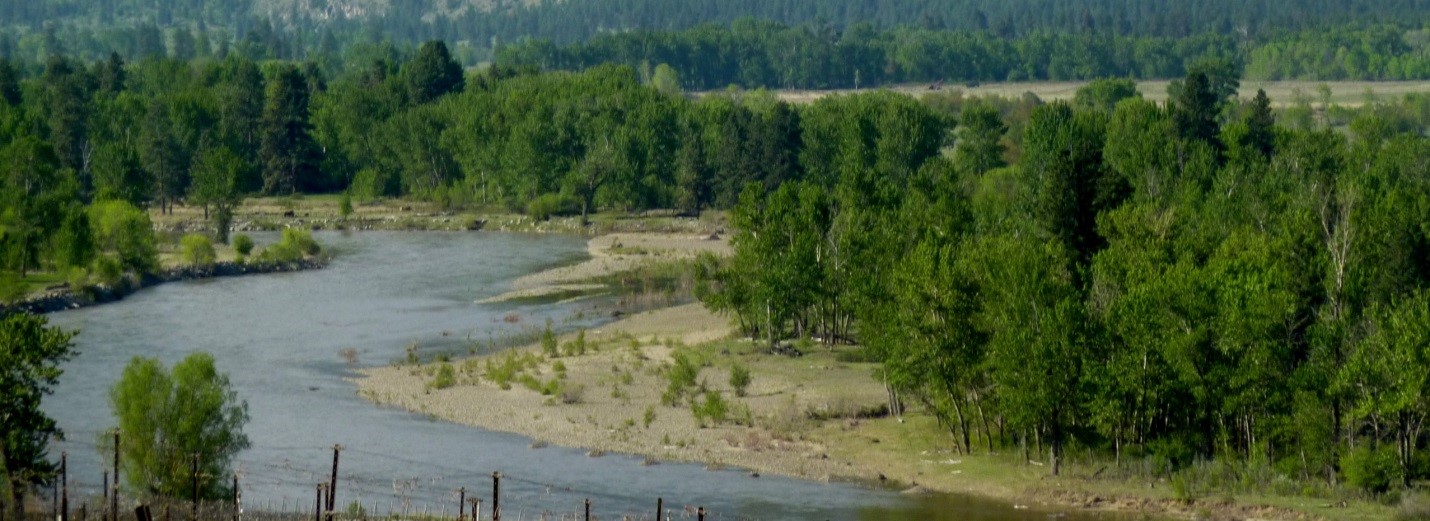
© Steven Earle. Used with permission.
Table 6.2 in the textbook summarizes the various types of clastic sedimentary rocks. Mudrock is dominated by silt- and clay-sized fragments, and if evidence of lamination (fine layering) exists, it is called shale (Figure 6.8 [a]). Mudrocks are deposited in very low-energy environments like lakes and the deep ocean.
Sandstone is dominated by sand-sized fragments (Figure 6.8 [b]) in several varieties, depending on the types of grains and the proportion of finer material. Arenite (see Figure 6.6) is clean sandstone with no more than 15% silt or clay. Almost all sandstones are dominated by quartz. If quartz is greater than 90%, it’s a quartz arenite. If less than 90%, and if more feldspar is present than rock fragments, it’s an arkose. If rock fragments (such as sand-sized grains of volcanic rock) outnumber feldspar grains, it’s a lithic arenite. The same diagram applies to silt/clay-rich sandstones called wackes (it’s a German word, pronounced “vack-e” but most students say “wacky”). Some sandstone examples are shown in Figure 6.7. Sandstones are deposited in a wide range of environments including rivers, deltas, beaches, the near-shore ocean, and deserts.
Coarse clastic sedimentary rocks are divided into two main types, depending on the roundness of the clasts. Granules and pebbles quickly become rounded if they are transported in water (e.g., a fast-flowing stream), and the resulting rock is called conglomerate (Figure 6.8 [c]). If the rock fragments are part of a slope failure—for example, if they tumble down a cliff—the rock is known as a breccia (Figure 6.8 [d]). Typically, conglomerates are deposited in high-energy rivers and sedimentary breccias (not to be confused with volcanic breccias) and are formed beneath steep cliffs.
Coal is deposited in swampy environments where the abundant dead organic matter can remain submerged in low-oxygen water for centuries or millennia until it is covered with more sediment. This typically happens on the floodplains of rivers that periodically change their course.
Completing Exercise 6.2 will help you to understand what is involved in describing and classifying sandstones and clastic rocks in general.
Chemical sedimentary rocks form when ions that have been transported in solution are precipitated as specific minerals or groups of minerals. Most limestone calcium (Ca2+) and carbonate (HCO3–) are taken from sea water by various types of organisms (corals, molluscs, algae etc.) and turned into calcium carbonate (e.g., the mineral calcite) that makes up shells and other structures (see Figure 6.9 and Figure 2-5). Fragments of these materials later accumulate to form rock limestone.
Enlarge
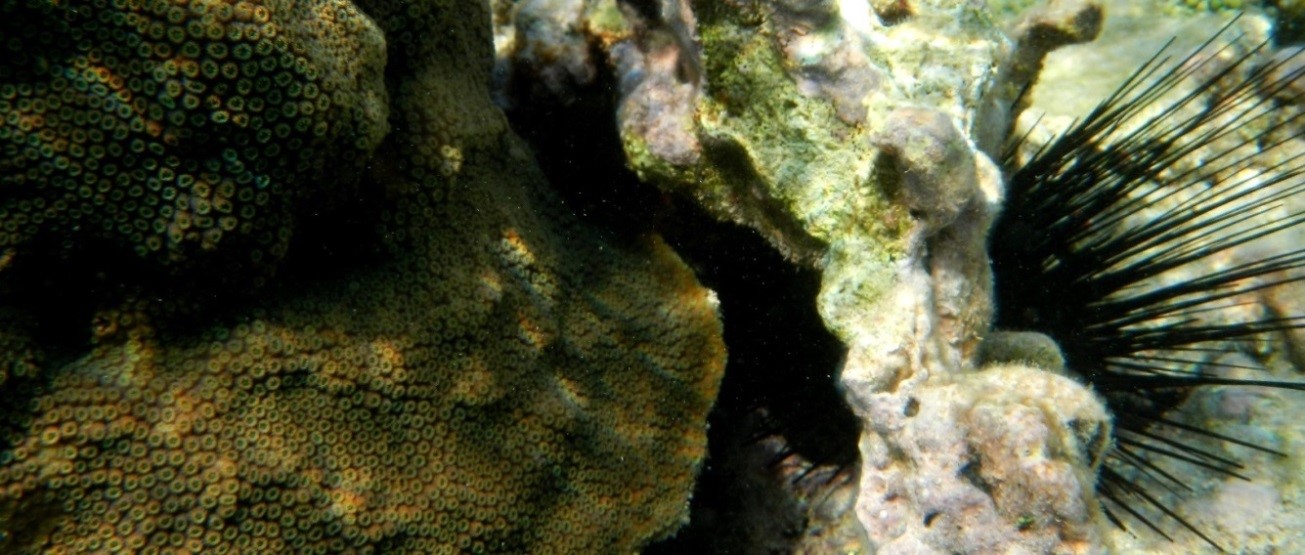
© Steven Earle. Used with permission.
Enlarge
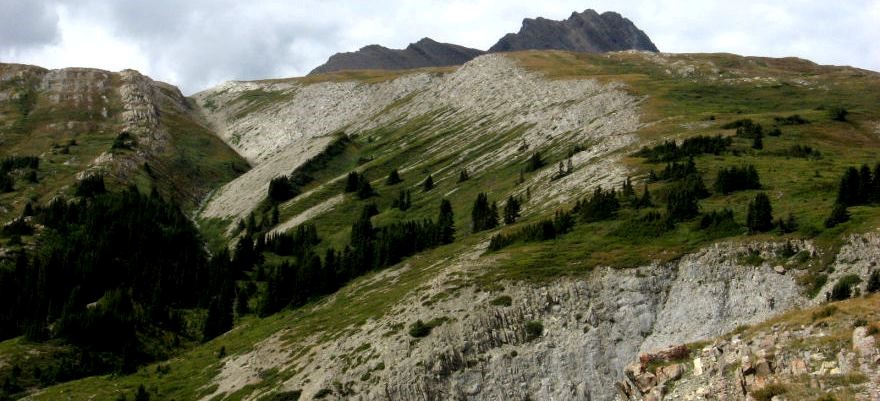
© Steven Earle. Used with permission.
Although most limestone forms from the calcium carbonate created by marine organisms, in some cases, the carbonate is precipitated directly from water by strictly chemical processes to form material such as the ooids shown in Figure 6.11 (c) or the tufa shown in Figure 6.12.
Many other types of chemical sedimentary rocks exist, and all of the important one are described and illustrated in the textbook. They include chert, banded iron formation, and evaporites. Please make sure you know how these types of rocks are formed. Note for registered students: You do not need to complete Exercise 6.3, since you did something similar in Assignment 1.
Section 6.3 deals with sedimentary depositional environments and sedimentary basins. Depositional environments include a wide range as is illustrated in Figure 6.17 and Table 6.3. It’s important to make the distinction between terrestrial and marine environments. Terrestrial includes all freshwater environments such as lakes, swamps, and streams, plus glaciers and deserts; whereas marine includes everything that’s within the oceans.
Exercise: Look carefully through the rows of Table 6.3 and make sure that you can see where each of these environments fits in Figure 6.17.
Most sediments that are deposited on land (e.g., in rivers or by glaciers) get re-eroded long before they can be turned into sedimentary rocks. Some of that re-erosion occurs within months or years, and sometimes it takes thousands of years, but even if its tens of thousands of years, that’s generally not long enough for the sediments to be lithified. Lithification, which is described briefly in Section 6.1, includes a range of processes that include the compression of sediment (from the weight of overlying sediments) such that all of the grains are touching, and the formation of cementing agents between the grains. These cements can include calcite, quartz, hematite, clay minerals, and various others, and it doesn’t take a lot of them to turn compacted sediment into hard rock, as is shown in Figure 2-7.
Enlarge
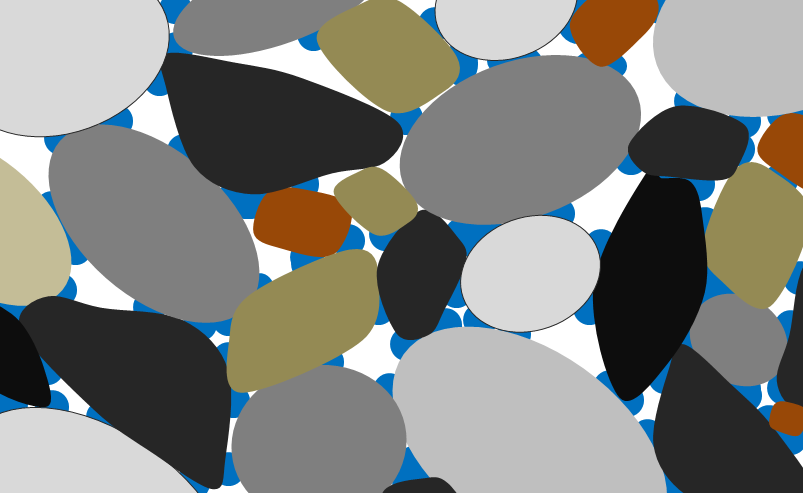
© Steven Earle. Used with permission.
To be preserved and become sedimentary rocks, sediments have to last long enough to be buried deep beneath other sediments. This process almost always occurs within some type of basin, and the process takes millions to tens of millions of years. Most such basins are created by tectonic processes when parts of the crust get pushed up or pulled down during plate interactions. Some of the tectonic situations where this occurs are illustrated in Figure 6.18. As noted previously, the Strait of Georgia is a forearc basin (Figure 2-8). The Cretaceous Nanaimo Group sedimentary rocks of Vancouver Island, which are described in Section 6.5, were deposited in a forearc basin.
Enlarge
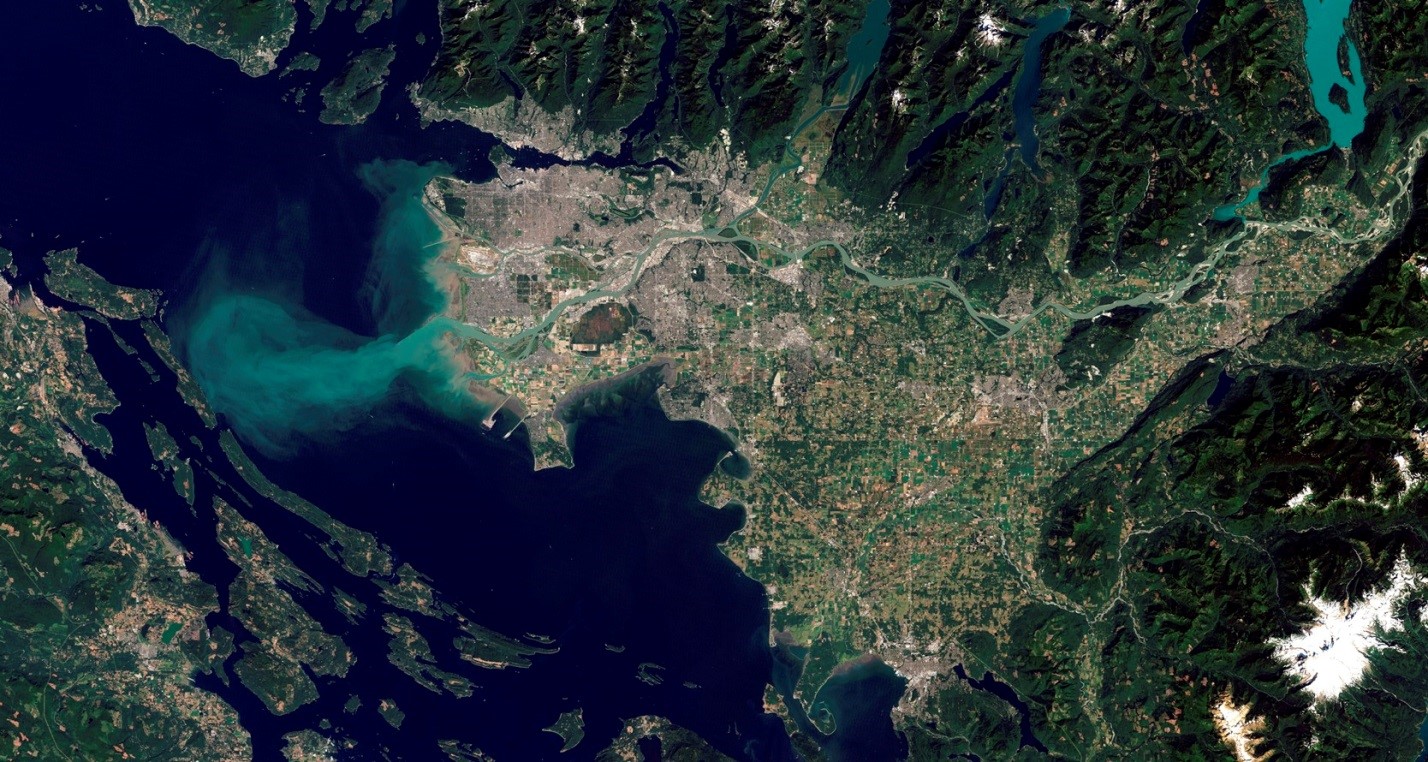
Simmon, R. & Allen, J. (2011). The big muddy, Western edition [Digital photo]. NASA's Earth Observatory. Retrieved from: http://earthobservatory.nasa.gov/IOTD/view.php?id=77368.
The following exercise should give you some practice in understanding the relationships between plate tectonics and the formation of sedimentary basins. Your Open Learning Faculty Member can check your answers or give you some help if you need it.
Exercise: Two sedimentary basins are depicted on the following perspective diagram. For each, indicate what type of basin it is and describe the mechanism of its formation.
Section 6.4 includes a discussion of sedimentary structures and fossils, but it starts with a summary of the important principles that can be applied to the study of sedimentary rocks. These principles are illustrated in Figure 2-9. Make sure that you understand what they mean, and more importantly, how they can be applied to understanding sedimentary rocks.
Enlarge
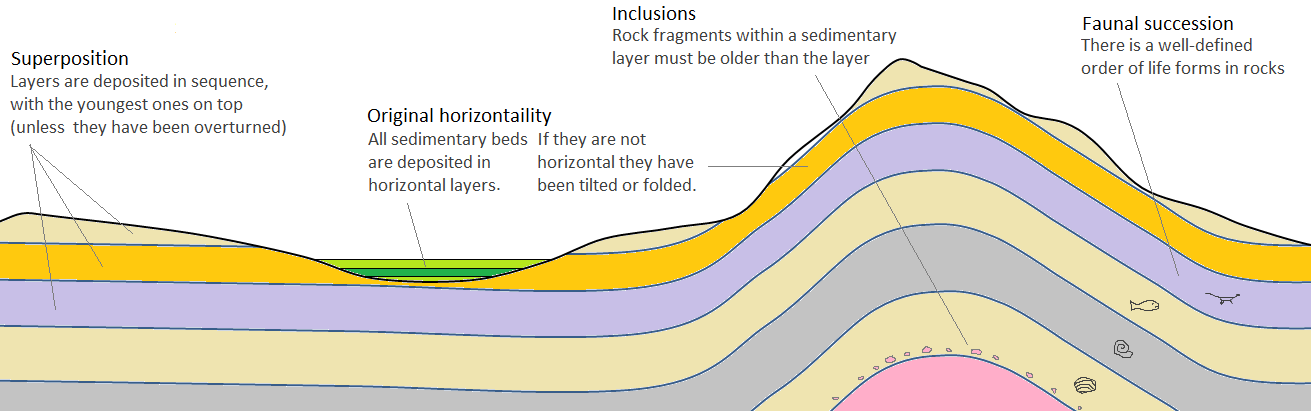
© Steven Earle. Used with permission.
Section 6.4 also includes a discussion of the importance of sedimentary structures to understanding sedimentary rocks and their depositional environments. Sediments and sedimentary rocks provide us with an extremely long (over 3 billion years) record of the conditions on the surface of the Earth, which includes the conditions under which existing rocks were weathered, the types and distances of sediment transportation, the nature of the depositional process and the environment in which deposition occurred, and even the climate at the time.
Read Section 6.4 and then complete Exercise 6.4 to make sure that you understand how you can interpret these types of features.
In section 6.5, we look at the systematic organization and naming of geological units. For the most part, this only applies to layered units—particularly to sedimentary rocks but also volcanic rocks, especially where they are interlayered with sediments. Make sure that you understand the hierarchy of classification in which the top level is known as a group, and that groups can be divided into formations, formations into members, and in a few cases—where particularly detailed geological mapping has been carried out—members into beds.
Intrusive igneous and metamorphic bodies are not normally named using the group-formation-member convention. Instead, it is common to call an intrusive body a stock, batholith, or complex (for example, the Coast Range Plutonic Complex). A body of metamorphic rocks is typically also called a complex.
The example used in the text to illustrate the naming of geological units is the Cretaceous Nanaimo Group, which underlies much of the eastern edge of Vancouver Island, extends beneath the Strait of Georgia, and also is present in a few locations on the mainland. The sediments that make up these rocks were deposited in a forearc basin that was the precursor to the existing Strait of Georgia forearc basin.
Please answer the questions at the end of Chapter 6, and then check your answers in the textbook.
2-3 Metamorphic Rocks
Metamorphism and metamorphic rocks, which are described in Chapter 7 of your textbook, occupy the bottom part of the rock cycle, and represent the processes that occur between the lithification of sedimentary rocks and the partial melting that forms the magma for igneous rocks. The transition between lithification and metamorphism occurs where some of the original minerals of the sedimentary or igneous “parent” rock become unstable (owing to elevated temperatures), and new minerals start to form.
Section 7.1 summarizes the factors that control metamorphic processes. The mineral composition of the parent rock determines which elements are available to make new minerals (see Figure 7.3). The temperature and pressure determines which existing minerals will become unstable and which new ones will become stable during metamorphism. The existence of directed pressure affects the texture of the new rock (see Figure 7.3). Fluids (especially water) are important because they can facilitate the movement of ions within the crust, and also can promote the growth of new minerals. Finally, time is important because metamorphic processes are amongst the slowest of any geological processes.
Completing Exercise 7.1 will give you an understanding of the amount of time necessary for metamorphic processes.
As described in Section 7.2, the first criterion in the classification of metamorphic rocks is their texture. Some metamorphic rocks are foliated—meaning they have planar fabric—and some are not foliated. Typically, the development of foliation depends on the existence of differences in the intensity of pressure in different directions, which normally is a product of plate convergence. Although some foliation forms simply because the rock is compressed more in one direction than in others (Figure 7.5), most foliation is a result of the preferential alignment of newly-formed minerals in a direction perpendicular to the main pressure direction (Figure 7.6). Planar minerals like mica and elongated minerals like amphibole are particularly important in imparting foliation to a rock because they grow with their long axes aligned.
An example of this process is shown in Figure 2-10 in which the crystals of mica have been forced to grow perpendicular to the main stress direction (pressure from the top and bottom in this view). Although the quartz and garnet formed under the same pressure conditions, these minerals are not elongated, and so they do not express the same degree of alignment.
Enlarge
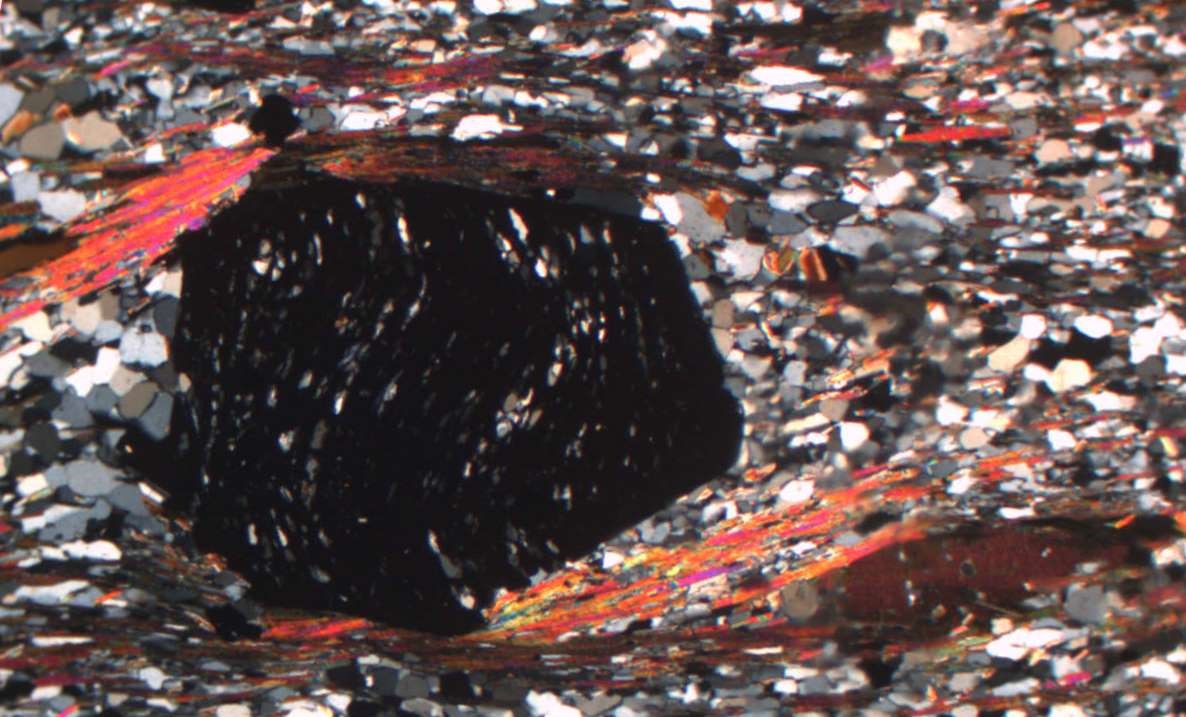
Dann, J. (2010). Thin section image of Garnet Mica Schist from Salangen, Norway (Digital image). Wikimedia Commons. Retrieved from: https://commons.wikimedia.org/wiki/File:Thin_section_of_garnet-mica-schist.jpg
Section 7.2 describes the textures and mineralogy of the four main types of foliated metamorphic rock (slate, phyllite, schist and gneiss). Make sure you are familiar with these.
Non-foliated metamorphic rocks are classified typically on the basis of their composition. They include marble (derived from limestone and consisting of primarily of calcite), quartzite (derived from sandstone and consisting primarily of quartz), and hornfels (derived from mudstone or fine sandstone and consisting of a range of different minerals, including micas and quartz).
As shown in Figure 7.12, some rocks that appear to be non-foliated may actually be foliated at a microscopic scale. This is quite common in quartzite and marble, and is an important indicator of the conditions under which the metamorphism occurred.
Complete Exercise 7.2 to gain some practice in interpreting the characteristics of metamorphic rocks.
Section 7.3 includes a discussion of the relationships between plate tectonics and metamorphism. As summarized in Figure 7.14, sedimentary or igneous rocks that formed under one set of pressure and temperature conditions may be subjected to different conditions as a result of plate interactions. Under these different conditions, some of their minerals are no longer stable. For example, where continents collide and mountain ranges are formed (Figure 7.15), sedimentary rocks—which are comprised of minerals that formed under surface conditions (such as clays) —can be buried to depths of tens of kilometres and subjected to temperatures of several hundred degrees. Make sure you understand this environment of metamorphism, and also that which occurs near to sea-floor spreading ridges (Figure 7.16), and the contact metamorphism that occurs in the vicinity of an upper-crustal magma chamber (Figure 7.19).
Contact metamorphic zones (or aureoles) tend to be only metres to tens of metres in extent. In contrast, regional metamorphism, related to burial beneath mountain ranges and heating at spreading ridges, affects large volumes of rocks measured in thousands of cubic kilometres. As a result, the vast majority of metamorphic rock is formed under conditions of regional metamorphism, and most of it is foliated.
The geothermal gradient (the rate of increase in temperature with depth) differs in different geological environments. In a subduction zone, for example, the cold descending oceanic crust does not heat up as quickly with depth as does the continental crust in other areas. Conversely, in areas of volcanic activity, it’s possible to encounter hot rocks at much shallower depths than elsewhere. These differences in the geothermal gradient result in a wide range of pressure-temperature conditions within the crust, as is illustrated in Figure 7.20. Take a careful look at this diagram to assist you in visualizing where some of the different types of metamorphic rocks form, but don’t feel that you need to memorize these relationships. Completing Exercise 7.3 will help you to understand Figure 7.20.
Section 7.4 describes regional metamorphism in more detail and introduces the concept of metamorphic index minerals. Figure 7.21 shows some of the important index minerals in the context of temperature, but don’t forget that pressure also plays an important role in mineral stability, as we saw with respect to the minerals kyanite, andalusite, and sillimanite in Figure 7.3.
The processes commonly related to regional metamorphism—including continental collision and mountain building; thickening of the continental crust and isostatic adjustment of the crust-mantle boundary; development of metamorphic zonation related to burial depth; and finally erosion, uplift, and isostatic readjustment—are illustrated in Figures 7.22 and 7.23, which use an example from Nova Scotia. Make sure that you understand how crustal thickening and differential crustal rebound following erosion play critical roles in determining the distribution of metamorphic zones in this situation. Exercise 7.4 may help you to understand these processes.
As described in Section 7.5, contact metamorphism occurs adjacent to igneous intrusions at relatively shallow depths in the crust. Differential pressure is not normally a major factor at shallow depths, so foliation is uncommon. Typical rock types formed include marble, quartzite, and hornfels. Complete Exercise 7.5 to reinforce your understanding of these rock types and processes.
Upper crustal intrusions have significant impacts on the distribution of heat near to the Earth’s surface, which can lead to the development of convection systems within groundwater. In addition, a great deal of water can be given off by a cooling body of magma, and when this water is dispersed into the surrounding rock, it can have significant effects on other surrounding rocks. These processes are illustrated in Figure 7.25 and described in the associated text. Several important types of ore deposits, including many of those found in British Columbia, can be formed under these conditions.
You are encouraged to answer the questions at the end of Chapter 7, and then check your answers in the textbook.
2-4 Geological Time
Chapter 8 in your textbook examines the various ways of measuring geological time. In Section 8.1, we look at how geological time is divided into manageable chunks, with names that are used around the world. Read through the description of the Geological Time Scale. You don’t need to know the names and time limits of the eons, eras, periods, epochs, and ages, but you should be aware that the last 542 Ma* is known as the Phanerozoic (Figure 8.4), and that it is divided into Periods, the names of some of which you may already be familiar, such as Cambrian, Devonian, Jurassic, Cretaceous, and Quaternary. Currently, we are in the Quaternary Period, which started at 2.6 Ma.
*Remember from Unit 1 that Ma means millions of years before the present, so 542 Ma means 542 million years ago.
In Section 8.2, we consider relative dating methods, including the use of inclusions and crosscutting relationships to determine the relative ages of rocks. These methods are in addition to the principles of superposition and faunal succession that were discussed earlier in this unit. Figure 2-11 shows a simplified cross-section of a series of sedimentary and igneous rocks. The order in which these were deposited, and the rationale for determining that order, are summarized in Table 2-1 that follows.
Enlarge
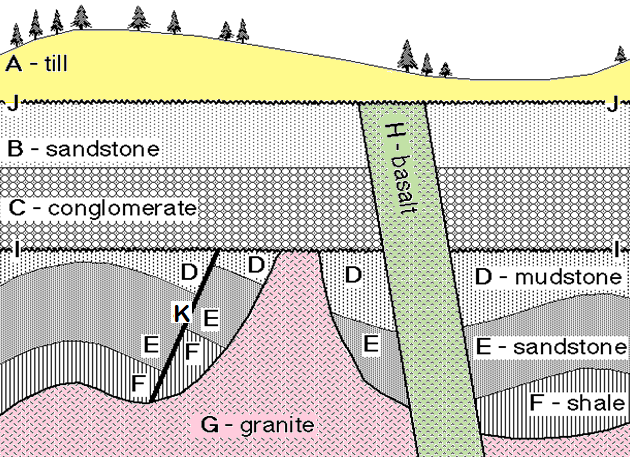
© Steven Earle. Used with permission. (Modified from an existing GEOL-1011 exam, original by Dr. David Huntley)
Order | Feature | Explanation |
---|---|---|
11 | A – till | This glacial deposit overlies everything and is not cross-cut by anything. |
10 | J – unconformity | The rocks below this line were eroded to a flat surface prior to (or during) the deposition of A. |
9 | H – basalt | The basalt dyke cuts across everything else (except A and J), so it must be younger. |
8 | B – sandstone | The sandstone overlies the conglomerate, so it must be younger. |
7 | C – conglomerate | The conglomerate overlies all of the rocks beneath and is cross-cut only by the basalt dyke. |
6 | I – unconformity | The underlying features (D, G, and K) have been eroded to a flat surface that cuts across all of them. |
5 | G – granite | The granite has intruded through all of the remaining features (D, E, F, and K), so it must be younger. |
4 | K – fault | The fault cuts across D, E, and F, so it must be younger than them. |
3 | D – mudstone | The mudstone overlies the sandstone, so it must be younger. |
2 | E – sandstone | The sandstone overlies the shale, so it must be younger. |
1 | F – shale | The shale is the lowest and oldest of the sedimentary layers. |
Section 8.3 describes the concepts related to dating rocks using fossils. The underpinning of this technique is the Principle of Faunal Succession that is illustrated on a large time scale (the latest Proterozoic and all of the Phanerozoic) in Figure 8.10. Over that time scale occurred the evolution of all of the major large plants and animals, and the extinction of many important groups such as trilobites, ammonites, and dinosaurs.
In the early years of palaeontology, the identification of fossil assemblages only provided relative dates for different strata because the absolute ages of organisms were not known. However, since the early 20th century, geologists have been dating rocks using isotopic techniques, and it has been possible to assign actual dates to the age ranges of different fossil species. For some organisms, these age ranges are relatively narrow (e.g., less than a million years), whereas for others, they are several to even tens of millions of years. However, even where the ranges are long, it is common for a layer of rock to have several different fossils whose age ranges overlap, and thus the age of the rock can be quite well constrained, as illustrated in Figure 8.11.
You can practice narrowing down ages using fossil data in Exercise 8.2.
Section 8.3 also includes some discussion on the application of index fossils and biozone fossils for constraining the ages of rocks. An important feature of a good index fossil is that the species lived over a wide area, and thus can be used to correlate rock ages across several continents.
Isotopic dating (Section 8.4) depends on the fact that certain radioactive isotopes decay at a consistent and precisely known rate, and that by doing a chemical analysis of a rock or mineral sample, we can determine the proportions of the parent isotope (for example, potassium-40) and the daughter isotope (argon-40) to estimate the age of the sample. For results to be useful, we have to be confident that none of the daughter isotope was present in the rock or mineral when it was formed, and that none of the parent or daughter isotopes have been removed since the time of formation. Geologists have developed sampling techniques to ensure that this is the case.
Isotopic dating, best applied to igneous rocks, can tell us the time at which the magma cooled and crystallized. It also can also be used with metamorphic rocks, but, in this case, we would be dating the time of metamorphism, rather than the formation of the original rock. As described in the textbook, isotopic dating cannot be applied to sedimentary rocks because they are made up of a variety of materials that are all older than the age of the rock. We can apply isotopic methods indirectly to dating sedimentary rocks if they are interbedded with volcanic rocks or if they are cut by igneous dykes, although in the latter case, we would only be able to say that the sedimentary layers are older than the dyke.
Complete Exercise 8.3 to get some practice with isotopic dating, and add this extra question: If the granite in question was in the form of a dyke that cut across some sedimentary rock layers, what could we say about the age of the sedimentary rock?
Section 8.5 describes a number of other methods for dating geological materials, and several more that are not included in this Unit. As we’ll see in the next unit, magnetic dating techniques are very important with respect to plate tectonics. Exercise 8.4 will give you some experience with using these techniques.
Section 8.6 includes a discussion of the importance of having a real understanding of geological time. This knowledge is critical for anyone who wants to put past events into perspective, such as: What is the relationship between glaciation and human evolution and migration? Or how and why has the climate changed in the distant past? Or when did birds evolve?
Carefully read Section 8.6, and then complete Exercise 8.5.
Then, answer the questions at the end of Chapter 8, and check your answers in the textbook.
This completes the notes for Unit 2.
Note if you are a registered student: If you haven’t already done so, now is the time to start working on Assignment 2 (go to “Assignments” on the course Home Page). You should find most of what you need to complete this assignment in these unit notes and Chapters 5 to 8 of your textbook, but you might also need to refer to other sources of information.